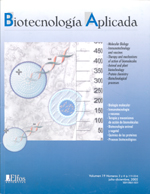
|
Biotecnologia Aplicada
Elfos Scientiae
ISSN: 0684-4551
Vol. 13, Num. 1, 1996, pp. 1-7
|
Biotecnologia Aplicada 1996;13:1-7
VACCINES AGAINST Neisseria meningitidis: PAST, PRESENT AND
FUTURE
Dlawer A. A. Ala'Aldeen.
Division of Microbiology, Department of Clinical Laboratory
Sciences, University Hospital, Queen's Medical Centre, Nottingham
NG1 2UH, United Kingdom.
Recibido en septiembre de 1995. Aprobado en octubre de 1995
Code Number: BA96001
Size of Files:
Text: 50.8K
No associated graphics files
SUMMARY
The ultimate goal in meningococcal vaccine research is the development
of an ideal vaccine which is safe, offers long lasting immunity
to all age groups, cross-protects against all meningococcal serogroups,
serotypes and serosubtypes, be given orally or nasally and be
easily incorporated into the World Health Organisation's Expanded
Program on Immunisation. So far no such vaccine has been developed.
Vaccines based on the capsular polysaccharides are available against
serogroups A, C, W135 and Y which offer good, but relatively short-lived,
protection against their respective serogroups. These vaccines
do not cross-protect against serogroup B meningococci. Various
alternative approaches have been now explored, including improved
capsular polysaccharides and preparations of outer membrane proteins
(constitutively expressed or iron-regulated). Over the past few
years there has been considerable activity in the field and a
number of clinical trials were conducted on various vaccine preparations
with varying successes. We may witness research breakthroughs
in the foreseeable future, however, it may be some time before
a broadly or universally cross-protective vaccine becomes available.
Key words: Meningococci, vaccines, iron, iron-regulated proteins,
transferrin, transferrin-binding proteins, outer membrane proteins,
capsule, polysaccharide, immune response
RESUMEN
La vacuna antimeningocóccica ideal debe ser segura, conferir
una inmunidad duradera en todos los grupos etarios, proteger contra
todos los serogrupos, serotipos y serosubtipos de Neisseria meningitidis,
administrarse oral o nasalmente, y poder ser incorporada con facilidad
dentro del Programa Ampliado de Inmunización de la Organización
Mundial de la Salud. Hasta ahora tales objetivos no se han alcanzado.
Existen vacunas basadas en el polisacárido capsular contra
los serogrupos A, C, W135 y Y, las cuales ofrecen una buena, aunque
relativamente corta, protección contra sus serogrupos respectivos.
Éstas no ofrecen protección cruzada contra el serogrupo
B. Para lograrlo se han explorado varios enfoques alternativos,
incluyendo polisacáridos capsulares mejorados y preparaciones
de proteínas de membrana externa (de expresión constitutiva
o reguladas por hierro). Ha habido una considerable actividad
en este campo durante los últimos años y varias
preparaciones vacunales han sido llevadas a pruebas clínicas
con diferentes grados de éxito. Aunque es posible que presenciemos
adelantos cardinales en este campo en el futuro cercano, puede
pasar algún tiempo antes de que dispongamos de una vacuna
antimeningocóccica de amplio espectro de protección.
Palabras claves: Meningococci, vacunas, hierro, proteínas
reguladas por hierro, polisacárido, transferrina, proteínas
de membrana externa, proteínas de unión de transferrina,
cápsula, respuesta inmune
Introduction
Neisseria meningitidis (meningococcus) is the commonest
cause of pyogenic meningitis and is the only bacterium that is
capable of generating epidemic outbreaks of meningitis. The pathogenesis
of the disease remains unknown, however, it is known that the
organism colonises the nasopharynx by adhering to the non-ciliate
columnar cells. It will then reach the sub-epithelial cells and
finally the blood stream where, if survived, it may cause bacteræmia
and cause a number of different clinical syndromes depending on
the host's immunity and a number of other unknown factors. The
syndromes can vary in severity from a transient mild flu-like
illness to fatal meningitis or septicæmia. Mortality can
vary between 10-30 % depending on socio-economic factors e.g.
the standard of health care.
However, in Europe and North America up to 14 % of the patients
die despite the high standard of living and health care in these
two continents and despite the sensitivity of the organism to
many antibiotics. Furthermore, of those patients who recover,
a significant number will develop permanent neurological sequelae,
such as cranial nerve deficits.
Epidemiology
Currently, we are experiencing a world-wide epidemic with a clear
increase in the number of cases reported in the recent years.
In the United Kingdom, e.g., more than 1 300 cases/year were reported
in the past few years and around 60 % of these occurred in children
under five years of age, 40 % of which were children aged less
than one year (1). In the United States, approximately 2 600 cases
of meningococcal disease have occurred annually over the past
few years with a case-fatality rate of 12 % (2) and 46 % of the
of cases affect those of two years of age or younger. In third-world
countries, it is estimated that more than 0,3 million cases occur
each year with up to 30 % fatality. In the meningitis belt of
Savanna Africa, attack rates reach up to 1 000 cases per 100 000
population In this region, epidemics occur every five to ten years
and last for approximately 2-4 years (3), but, in contrast to
other areas, cases occur mainly in the hot dry months. Large scale
epidemics have also occurred in many countries of Asia (e.g. Pakistan,
India and China), and Central and Latin America (e.g. Cuba, Chile
and Brazil). In Cuba, the attack rate reached levels of more than
50 cases per 100 000 population in children younger than 6 years
(4).
Based on the antigenic differences in their capsular polysaccharide
(CPS), 13 serogroups of N. meningitidis have been identified,
with Groups A, B and C responsible for 90 % of the cases. Group
A meningococci are now rare in the more developed countries, but
are the major pathogen in the meningitis belt of Africa and a
number of Asian countries. In Europe, South Australia and the
New world (North, Central and Latin America), Group B is responsible
for the vast majority of cases followed by Group C (4, 5, 6, 7,
8).
N. meningitidis is further classified immunologically
into serotypes, serosubtypes and immunotypes, based on antigenic
differences in class 2/3 outer membrane proteins, class 1 outer
membrane protein and lipooligosaccharides respectively. Many of
these antigens are considered as vaccine candidates.
It is interesting that within one geographical location there
is a trend for each serotype and subtype, particularly among serogroups
B and C, to change with time. For example, Group B:15:Pl.7.16
strains have been found responsible for infections in England
and Wales. However, there are currently more serologically non-typable
strains isolated in these two countries than any individual types
identified (1, 9), reflecting the emergence of new strains. These
changes clearly will have important implications for the design
of vaccines based on serotype and subtype outer membrane proteins.
In this context, it is interesting to note that the class 1 protein,
product of the porA gene (10), is known to be expressed by most
but not all meningococcal isolates (11, 12, 13). Furthermore,
with increased international travel, global dissemination of
outbreak-associated strains is common.
Correlates of Protection
Despite extensive studies over the past few decades, the mechanisms
responsible for the development of natural immunity against meningococci
remains unclear. Protection has been correlated with the presence
of bactericidal antibodies (14) and following the study reported
by Goldschneider and colleagues (15) bactericidal assays have
become established as the best available test to determine the
protective ability of specific antisera raised against vaccine
candidates.
However, it is not certain to what extent the in vitro experimental
conditions reflect events occurring in vivo nor whether
the above data apply to infections with all serogroups. The data
linking bactericidal antibodies with protection relate primarily
to the Group A and C polysaccharides but have been extended to
include bactericidal antibodies against outer membrane proteins.
While the great majority of the studies have focused on the role
of serum bactericidal activity in the host's defense against meningococcal
disease, much less attention has been given to the cellular immune
response (e.g. helper T-cell) and killing of bacteria by phagocytosis,
and therefore little is known about the importance of phagocyte-mediated
killing of meningococci as compared to serum bactericidal activity.
The Capsular Polysaccharides (CPS)
Group A and C capsular polysaccharide
The first successful vaccines produced were against N. meningitidis
Group A and C using high molecular weight (100 kDa) CPS
of these strains (15). A series of large scale field trials were
conducted in the 1970s among different age groups in different
parts of the world, including Europe, Africa and Latin America
(16, 17, 18, 19) which showed that the CPS-vaccine is effective
in controlling epidemics of Group A disease in almost all age
groups. It soon became clear that antibody responses among infants
to the Group A and C CPS vaccines depended on a number
of factors including the age of the infant, the molecular weight
of the antigen, the number of doses of antigen, and the prior
experience of the infant with naturally occurring antigens cross-reactive
with the meningococcal CPS. It became evident that children under
the age of two years do respond, particularly to A CPS, with small
increases in specific antibodies.
The strength of the response and its duration increased with age
and in adults 100 % seroconversion was achieved which lasted longer
than in children (19, 20, 21, 22, 23, 24). For example, Reingold
et. al. showed that the Group A CPS vaccine efficacy in
children vaccinated at less than four years of age almost disappeared
over the following three years, whereas those who were four years
of age or older when vaccinated showed evidence of vaccine-induced
clinical protection for three years after vaccination (24).
The Group C CPS vaccine when administered routinely among recruits
of the United States army to prevent severe outbreaks, has virtually
eradicated the Group C meningococcal disease in this population.
Subsequent trials and antibody response studies among children
confirmed this success among age groups above two years but not
among children under two years of age (25, 26, 27, 28). It is
interesting that more recent data show that although anticapsular
antibodies and bactericidal activity in adults decline substantially
by two years following vaccination, both persist at a level significantly
above prevaccination levels for up to 10 years (29).
On the basis of these results, C CPS vaccines are now recommended
for general use in epidemics except for children under the age
of 2 years. In contrast, A CPS vaccines are recommended to be
given during epidemics in all age groups including infants with
a booster dose given to those under the age of 18 months. However,
it is important that, due to significant shortcomings, these CPS
vaccines are not useful for routine immunisation of infants. Production
of improved CPS vaccines, particularly against Group A and C meningococcal
infections, is still a research priority. A vaccine capable of
inducing protective immunity among infants is needed so that it
can be added to the routine childhood immunisation as part of
the Expanded Program on Immunisation.
The success of linking Hib CPS to a protein carrier which results
in a vaccine which induces a thymus-dependent (T-cell dependent)
IgG response in young children, and thus immunological memory,
has encouraged the development of CPS-protein conjugate vaccines
for serogroups A and C meningococci. Considerable work has therefore
been done to couple A and C CPS to proteins so as to change the
character of the antigen from thymus independent to thymus dependent
(30). These conjugates are generally strong immunogens and capable
of inducing memory (30, 31).
Conjugates of Group A and C CPS conjugated to tetanus toxoid or
a non-toxic mutant of diphtheria toxin have been shown to be highly
immunogenic in mice and rabbits. Conjugate vaccines against Group
A and C meningitis have been evaluated in toddlers by the NIAID
in the USA, and in Gambian infants by the Medical Research Council
(28, 32, 33). Results from these trials are expected in the near
future. The World Health Organisation's Steering Committee on
Encapsulated Bacteria: Program for Vaccine Development, is now
coordinating and facilitating the development of conjugate vaccines
against N. meningitidis, particularly serogroup
A, and has encouraged manufacturers to produce conjugates for
evaluation (34). The program is also coordinating projects to
standardise immunoassays to enable proper assessment of the antibody
responses to CPS vaccines, the evaluation of various clinical
trials and to allow comparisons of different vaccines, different
vaccination schedules, and different populations.
Group B capsular polysaccharide
Group B meningococcal CPS consists of repeated residues of alpha-
(2-8)-linked oligomers of sialic acid, 2-8-alpha-N-acetylneuraminic
acid, which serves as an important virulence factor and protective
antigen for the organism. Candidate vaccines based on the native Group
B polysaccharide (B CPS) induce a transient antibody response
of predominantly IgM isotype. This poor immunogenicity of the
Group B CPS, could be due to sensitivity to neuraminidases or
immunotolerance of the host due to its similarity to sialic acid
moieties in human brain tissues (35) which has caused considerable
concern regarding the possible induction in humans of adverse
autoimmune consequences by administration of B CPS based vaccines.
Nevertheless, attempts are on-going to produce a CPS-based Group
B vaccine.
It has been proposed that conformational determinants on the Group
B CPS as presented on the intact organisms, raise antibodies that
do not cross-react with the linear alpha-(2-8) linked determinants.
The conformational structure is therefore seen to be more important
in the generation of protective immunity than the primary polysaccharide
structure. Various ways of stabilising the molecule in order to
present an appropriate conformation, have therefore been investigated.
These include the formation of non-covalent complexes of B CPS
with OMPs, the binding to Al(OH)3 and the conjugation of the polysaccharide
to carriers such as tetanus toxoid (36, 37, 38, 39), and at best
only transient bactericidal B CPS specific antibodies of mostly
IgM class were detected.
Another approach to generate T-cell dependent protective IgG responses
has involved attempts to modify the structure of B CPS itself
prior to conjugation. By replacing the N-acetyl groups of the
sialic acid with N-propionyl groups, Jennings and colleagues produced
a highly immunogenic chemically modified form of Group B CPS (40).
The propionylated CPS when conjugated with tetanus toxoid yielded
T-cell dependent IgG response. It is interesting that two populations
of antibodies were generated in mice when tested, a population
reactive with isolated native B CPS with little bactericidal
activity, and another population non-reactive with B CPS but with
bactericidal activity against live organisms (41, 42). The latter
population of antibodies seems to recognise a conformational epitope
on the surface of the organisms which is probably formed by a
combination of more than one cell-wall element, including the
B CPS and, therefore, not present when the purified CPS is used
alone. Clearly considerable pre-clinical safety evaluations will
be necessary before such vaccines go into humans.
Outer Membrane Proteins (OMPs)
In view of the problems associated with the currently available
CPS vaccines and the poor immunogenicity of Group B CPS much of
the attention has been focused on non-capsular antigens, including
constitutively expressed and iron-regulated outer membrane proteins.
It is important to know that recurrence of meningococcal disease
is extremely rare in the absence of immunodeficiencies, irrespective
of the serogroups of the infective organisms. This indicates that
non-capsular antigens can generate long-lasting cross-protective
immunity.
Constitutively Expressed OMPs
Among the constitutively expressed OMPs, the class proteins (especially
class 1, 2 and 3 proteins) have attracted most of the attention.
These proteins show considerable interstrain antigenic variation,
hence used as a basis for the serotyping and subtyping scheme
for characterizing strains of N. meningitidis.
However, they are still considered attractive candidate vaccine
antigens because within a particular epidemiological setting the
majority of strains causing disease belong to only a limited number
of types and sub-types. Antibodies against the class l OMP as
well as the mutually exclusive class 2 and 3 OMPs have been detected
in both immunized and infected individuals, however, the presence
of antibodies does not necessarily correlate with protection (43,
44, 45). The class 4 OMP appears to be highly conserved
between meningococcal strains, however, it is thought to generate
antibodies that might block the effect of bactericidal antibodies
directed against other surface antigens (46, 47). Although details
of the blocking action, or its relevance in vivo in humans,
remain unclear, it has been proposed that future vaccines should
not contain this protein. Some have attempted to produce OMP vaccines
from class 4-mutants (46), and others have attempted to clone
and express the other class proteins in heterologous expression
systems such as or Bacillus subtilis (48, 49). The class 5 proteins,
which have also been considered as vaccine antigens, are known
to be surface exposed and induce antibodies in humans, however,
they undergo phase variations and, therefore, the protective value
of their antibodies is questionable.
An obvious drawback of vaccines based entirely on serotype and
serosubtype antigens is the fact that the predominant types and
subtypes associated with the disease in any one area change from
time to time. The immune-pressure created by these vaccines may
also contribute to such change. Therefore, it may be necessary
to include several serotype/subtype proteins in one vaccine. Attempts
have been made to address this for the class 1 protein by constructing
a vaccine strain of N. meningitidis capable of expressing more
than one subtype epitope of this protein (50, 51).
Another approach, adopted by McCarvil et al, includes expressing
variable but surface-exposed epitopes of the class 1 protein on
the surface of Escherichia coli (52). They have cloned in frame
the identified sequences into the lamB gene of the E.coli expression
vector pAJC 264. carrying these constructs expressed hybrid lamB
proteins containing the surface loops of N. meningitidis class
1 protein. If serotype/subtype based vaccines are indeed type
specific in humans then whichever way is chosen to overcome the
problem there will be a need for continuous detailed epidemiological
surveillance of disease-associated organisms in order to predict
the optimal vaccine composition for any given time and place.
In the past decade, a number of serogroup B meningococcal vaccines
based on serotype/subtype protein-enriched outer membrane proteins
were developed and tested in clinical trials (4, 7, 53). Following
large scale placebo-controlled, randomised double-blind trials,
only the vaccines produced in Norway and Cuba showed significant
protective efficacy. The Norwegian vaccine consists of 25 mg/dose
lipooligosaccharide-depleted outer membranes from the Norwegian
epidemic strain of N. meningitidis, B: 15:P1.7. 16, with only
traces of meningococcal CPS. Each dose of the Cuban vaccine consists
of 50 g of Group C CPS mixed with 50 mg of lipooligosaccharide-depleted
outer membranes from a Cuban epidemic strain, B:4:Pl.15. In addition,
the Cuban vaccine has been described as containing other higher
molecular proteins, as well as class proteins (4). The Norwegain
vaccine, given to children aged 14-16 years, produced point estimate
of protective efficacy of 57 % after a 30 month follow up and
was, therefore, considered insufficiently effective for general
use (53). The Cuban vaccine, given to children aged 10-16 years,
offered an estimated point efficacy of 83 % after 16 months of
follow-up (4) and, as a result, the vaccine is now incorporated
into the routine childhood vaccination programme in Cuba. Although
the Cuban trial did not directly address efficacy in children
aged less than 10 years, follow up studies of the mass vaccination
have suggested that the overall protective efficacy based on vaccine
coverage and incidence of disease in children under six years
old is about 93 % (4). However, when the Cuban vaccine was tested
in a case-control study in Brazil, protective efficacy was reported
to vary with age. The vaccine was effective in children aged 4
years and older, but not in younger children (8).
In order to address some of the unresolved issues, such as differences
in efficacy between the Cuban and Norwegian vaccines, the duration
of protection versus the number of doses given, and in an attempt
to establish a sounder basis for the evaluation of new candidate
vaccines, as well as to accelerate the development of more effective
preparations, a multinational collaborative study, sponsored by
the World Health Organization, was undertaken in Iceland in 1992-1993.
This prospective, randomised, double-blind study compared the
reactogenicity, immunogenicity and serum bactericidal activity
elicited in 408 young adults by 2 or 3 doses of either the Cuban
or Norwegian vaccines.
A polysaccharide serogroup A/C meningococcal vaccine was used
as a control. Results showed that the Cuban and Norwegian vaccines
were similar but not identical in protein composition, containing
class l, 3 and 4, Opc and FrpB proteins. Class 5.5 protein was
identified in the Norwegian vaccine only (54, 55). Differences
were also noted between the stabilities of the two vaccines both
prior to and after adsorption onto carrier. The overall results
of the Icelandic study were disappointing (56). Despite extensive
studies, during and after the Iceland trial, the issues of discrepancy
between the Cuban and Norwegian vaccine trials have not yet been
resolved. Therefore, it is concluded that efforts to understand
the mechanisms by which these vaccines confer protection should
now be intensified and other tests for evaluating possible immunological
correlates of protection explored. Detailed analysis of these
aspects has been recently reviewed in greater depth by Ala'Aldeen
and Griffiths (l995) (57).
Transferrin receptors and other iron regulated outer membrane
proteins
This exciting field has expanded very rapidly over the past few
years and iron-regulated proteins have attracted considerable
attention as possible vaccine candidates. When grown under iron-restriction,
meningococci express several proteins which appear to be suppressed
(partially or totally) under iron-sufficient growth conditions.
Many of these proteins, which vary in terms of their molecular
mass and cellular localisation, are believed to be directly related
to iron-acquisition from the host's iron binding proteins and
other iron sources. These iron-regulated proteins include two
transferrin-binding proteins (Tbpl and Tbp2) (58, 59), a 105 kDa
lactoferrin-binding protein (60), a 37 kDa periplasmic iron-binding
protein (Fbp) (61), an 85 kDa haemoglobin-haptoglobin utilisation
protein (Hpu) (62), two RTX cytotoxin-related proteins (a 120
kDa FrpA and a 200 kDa FrpC) (63, 64) and a 70 kDa protein (FrpB)
of uncertain function (65, 66).
Among the iron-regulated proteins, the Tbps (Tbpl and Tbp2) have
attracted most of the attention as vaccine candidates. It is now
clear that the transferrin receptor is formed, partly or wholly,
by the transferrin binding proteins Tbpl and Tbp2. Ala'Aldeen
et al. produced the evidence linking the Tbps with biologically
functional transferrin receptors in live meningococci (67). Rabbit
antisera containing antibodies against the Tbps inhibited the
specific binding between transferrin and live meningococci. Theoretically,
it is possible that antibodies against Tbps might interfere with
the chelation and uptake of iron from transferrin, and thereby
inhibit the survival and growth of the organism in vivo. Recently,
Lissolo et al. (1995) (68) demonstrated the ability of
such antibodies to inhibit meningococcal growth in vitro when
transferrin was used as the only source of iron. More recently,
Pintor et al. demonstrated the ability of anti-Tbp antibodies
to inhibit the acquisition of radioactive iron from transferrin
by live meningococci (unpublished results). It is not clear whether
this effect is entirely mediated by the blocking of transferrin-binding,
iron-internalisation or both.
Tbpl is a c. 98 kDa transmembranal protein which varies in molecular
weight only marginally (+ c. 5 kDa) between meningococcal strains
(59, 69). This protein loses its biological and much of its immunological
properties when exposed to denaturing conditions, such as those
used for SDS-PAGE, and hence it is not visualised on Western blots.
Tbp2 (c. 65-90 kDa) shows considerable molecular and antigenic
heterogeneity amongst different strains of N. meningitidis (59,
70, 71) and retains its transferrin-binding activity and strong
immunogenic properties following SDS-PAGE. This protein is now
believed to be a lipoprotein anchored to the outermost layer of
the cell membrane (72).
Both Tbpl and Tbp2 proteins generate widely heterogeneous immune
responses in vivo, depending on the host species, Tbp2
isotype, the vaccine preparation, the route of administration
and other less well understood factors. The available data suggest
that Tbp2 possesses strain-specific and cross-reactive epitopes,
as determined by Western blots (59, 73). It has been demonstrated
that mice, infected with live organisms or vaccinated with natively
purified Tbps generate strain-specific anti-Tbp2 antibodies, whereas
similarly treated rabbits generate broadly cross-reactive anti-Tbp2
antibodies (59). It is interesting that humans recovering from
natural infection responded with fully cross-reactive anti-Tbp2
antibodies (59).
Rokbi et al., raised rabbit anti-Tbp antibodies using gel-extracted
Tbps obtained from two different strains, representing strains
with low (F kDa) and high (70 kDa) molecular weight Tbp2 molecules
(69). They clearly highlighted the presence of mutually exclusive
epitopes which divided the Tbp2 molecules into two different families
(groups, isotypes). This antigenic heterogeneity correlated well
with molecular and genetic heterogeneity. The majority of the
examined strains expressed high molecular weight Tbp2 and showed
full cross-reaction between them, but failed to cross-react with
the low molecular weight Tbp2 isotypes, Ferreiros et al.,(1994)
(74), using human convalescent sera, studied immunoreactivity
of Tbp2 molecules of different strains and obtained reaction patterns
which supported this isotype classification.
Danve et al.,(1993) (75) have shown that mice, whether actively
immunised with purified Tbps or passively immunised with rabbit
polyclonal anti-Tbp antiserum, protect mice from meningococcal
challenge with the homologous strain. They also showed that rabbit
anti-Tbp antibodies are bactericidal against some (not all) heterologous
test strains, irrespective of the strain identity in terms of
serogroup, serotype and serosubtypes. More recently, Lissolo et
al., (1995) (68) demonstrated that mice and rabbits immunised
with purified Tbp2 produced bactericidal antibodies capable of
protecting mice from lethal challenge. However, they failed to
purify native Tbpl and, therefore, failed to define the role of
this polypeptide in generating protective immunity.
More recently, Ala'Aldeen and Borriello have shown that murine
and rabbit anti-Tbp antisera (raised to natively purified Tbps)
kill homologous and heterologous meningococcal strains with no
obvious correlations between the bactericidal activity of the
antisera and the molecular mass or the Western blot profile of
Tbp2 (76). Animal antisera were able to kill strains which expressed
Tbp2 molecules of either higher or lower molecular weight isotypes.
Conversely, even strains expressing Tbp2 molecules of almost identical
mass showed some variation in susceptibility to the sera. Also,
they killed strains which showed no cross-reactivity on Western
blots and, conversely, some strongly cross-reactive strains were
not killed by these sera. These observations indicated that the
bactericidal antibodies were not restricted to those generated
against linear epitopes. While most of the generated anti-Tbpl
antibodies are directed to discontinuous epitopes, antibodies
to Tbp2 are to continuous epitopes, though some might also be
directed against conformational epitopes.
More recently, Bishop et al., have raised murine monoclonal anti-Tbpl
antibodies, one of which shows bactericidal activity and recognises
a conformational epitope, and another one reactive with a linear
epitope failed to kill the organism (77). Therefore, the Tbps
seem to possess a combination of important epitopes which can
generate protective antibodies capable of killing the organism
by complement mediated bactericidal activity and/or nutritional
starvation. It is possible that a mixture of more than one Tbp2
isotype (depending on the prevalent meningococcal strains in any
one country) with stabilised native Tbpl molecules will be required
in order to enhance and broaden the protective efficacy of any
Tbp-based vaccines.
Thus, it is now increasingly evident that both Tbps are surface-exposed
and immunogenic in humans and animals, and antibodies to their
native structure are bactericidal to homologous and many heterologous
strains. This suggests that a meningococcal vaccine based on,
or enriched with, undenatured Tbps from one or more strains in
combination with conventional CPS-based vaccines might increase
the spectrum of strains against which protection can be achieved
to include serogroup B strains.
CONCLUSIONS
It is evident that there has been considerable activity in the
meningococcal vaccine field over the past few years and we may
see breakthroughs in the foreseeable future. However, it may be
another decade or so before safety and efficacy data from large
scale clinical trials become available. It may take even more
time before a broadly or universally cross-protective vaccine
becomes available and/or incorporated into childhood immunisation
programmes. It is unfortunate that no reliable serological correlates
of protection exist, nor an universally accepted animal model
for estimating protecting potency. Measurements of serum bactericidal
activity, which proved useful as a correlate of protection assay
for CPS vaccines, may not be as useful in assessing the protection
afforded by OMP-based vaccines. Furthermore, studies with murine
monoclonal antibodies, Western blot analysis and current so called
animal models may be extremely misleading. Accumulating data suggests
that mouse and man respond differently to different meningococcal
antigens (59). No doubt, there is a greater need than ever before,
for more appropriate parameters for correlates of protection to
be found. Furthermore, in order to develop vaccines of consistent
composition and efficacy, more detailed analysis of future vaccines
will be required.
The OMP-based vaccines which have undergone clinical trials, so
far, are extremely complex preparations and it is not easy to
establish which of the numerous components really contribute to
protection in vaccinees. Also, greater knowledge on cellular immune
response (particularly, B-cell and T-cell responses) to meningococcal
antigens is required before T-cell dependent protective antigens
are tested and the ideal vaccine is developed.
- Jones DM and Kaczmarski EB. Meningococcal infections in England
and Wales: 1992. Com Dis Rep 1993;3:R129-131.
- Jackson LA and Wenger JD. Laboratory-bases surveillance for
meningococcal diseases in selected areas, United States. MMWR
1993;42:21-30.
- Lapeyssonie L. La meningite cerebro-spinale en Arfique. Bull
WHO 1963;28 (Suppl):3-114.
- Sierra GVG, Campa HC, Varcrcel MN,Garca IL, Izquierdo PL,
Sotolongo PF, Casanueva GV, Rico CO, Rodrguez CR and Terry MH.
Vaccine against group B Neisseria meningitidis: protection trial
and mass vaccination results in Cuba. NIPH Ann 1991;14:195-210.
- Frasch CE. Vaccines for prevention of meningococcal disease.
Clin Microbiol Rev 1989;2. Suppl: S 134-138.
- Hansman D. Meningococcal disease in South Australia: 1971
through 1989. In: Neisseria I990. Eds. Achtman M, Kohl P, Marchal
C, Morelli G, Seiler A and Thiesen B. Walter de Gruyter. Berlin
1991;71-74.
- Zollinger WD, Boslego J, Moran E, Garca J, Cruz C, Ruiz S,
Brandt B, Martnez M, Arthur J, Underwood P, Hankins W, Mays J,
Gilly J and TCNCf M. Disease. Meningococcal serogroup B vaccine
protection trial and follow-up studies in Chile. NIPH Ann 1991;14:211-212.
- De Moraes JC, Perkins BA, Camargo MC, Hidalgo NTP, Barbosa
HA, Sacchi CT, Gral IML, Gattas VL, Vasconcelos HG, Plikaytis
BD,Wenter JD and Broome CV. Protective efficacy of a serogroup
B meningococcal vaccine in Sao Paulo, Brazil. Lancet 1992;340:1074-1078.
- Jones DM and Kaczmarski EB. Meningococcal infections in England
and Wales: 1993. Com Dis Rep 1994;4:R97-R101.
- Barlow AK, Heckels JE and Clarke IN. The class 1 outer membrane
protein of Neisseria meningitidis: gene sequence and structural
and immunological similarities to gonococcal porins. Mol Microbiol
1989;3:131-139.
- Woods JP and Cannon JG. Variation in expression of class 1
and class 5 outer membrane proteins during nasopharyngeal carriage
of Neisseria meningitidis. Infect Immun 1990;58:569-572.
- Achtman M, Kusecek B, Morelli G, Eickmann K, Jianfu W, Crowe
B, Wall RA, Hassan-King M, Moore PS and Zollinger W. Comparison
of the variable antigens expressed by Clone IV-l and subgroup
III of Neisseria meningitidis serogroup A. J Infect Dis 1992;165:54-68.
- McGuinness BT, Lambden PR and Heckels JE. Class 1 outer membrane
protein of Neisseria meningitidis: epitope analysis of the antigenic
diversity between strains, implications for subtype definition
and molecular epidemiology. Mol Microbiol 1993;7:505-514.
- Frasch CE. Immunisation against Neisseria meningitidis. In:
Medical Microbiology 1983. Eds. Easmon C and Jeljaszewics J. Academic
Press. London. 115-144.
- Goldschneider I, Gotschlich EC and Artenstein MS. Human immunity
to the meningococcus-II. Development of natural immunity. J Exp
Med 1969;129:1327-1348.
- Erwa HH, Haseeb MA, Idris AA, Lapeyssonnie L, Sanborn WR and
Sippel JE. A serogroup A meningococcal polysaccharide vaccine.
Studies in the Sudan to combat cerebrospinal meningitis caused
by Neisseria meningitidis group A. Bull WHO 1973;49:301-305.
- Wahdan M, Rizk F, El-Akkad M, El Ghoroury A, Hablas R, N.
Girgis, A. Amer, W. Boctar, J. Sippel, E. Gotschlich and R. Triau.
A controlled field trial of a serogroup A meningococcal polysaccharide
vaccine. Bull WHO 1973;48:667-673.
- Makela, P. H., H. Kayhty, P. Wecksstrom, A. Sivonen and O.
V. Renkonen. Effect of group A meningococcal vaccine in army recruits
in Finland. Lancet 1975;8: 883-886.
- Peltola, H., P. H. Makela, H. Kayhty, H. Jousimies, E. Herva,
K. Hallstrom, A. Sivonen, O. V. Penkonen, O. Petty, V. Karanko,
P. Ahonen and S. Sarna. Clinical efficacy of meningococcus group
A capsular polysaccharide vaccine in children three months to
five years of age. New Eng J Med 1977;297:686-691.
- Gold, R., M. L. Lepow, I. Goldschneider, T. L. Draper and
E. C. Gotschlich. Clinical evaluation of group A and C meningococcal
polysaccharide vaccines in infants. J Clin Invest 1975;56:1536-1547.
- Lepow, M. L., I. Goldschneider, R. Gold, M. Randolph and E.
C. Gotschlich. Persistence of antibody following immunisation
of children with groups A and C meningococcal polysaccharide vaccines.
Pediatrics 1977; 60:673-80.
- Makela PH, Peltola H, Kayhty K, Jousimies H, Pettay O, Ruoslahti
E, Sivonen A and Renkonen OV. Polysaccharide vaccines of group
A Neisseria meningitidis and Haemophilus influenzae type b: a
field trial in Finl. J Infect Dis 1977;136:S43-50.
- Kayhty H, Karanko V, Peltola H, Sarna S and Makela PH. Serum
antibodies to capsular polysaccharide of group A Neisseria meningitidis
followed for three years in infants and children. J Gen Microbiol
1980, 135:851-863.
- Reingold AC, Broom CV, Hightover AW, Ajello GW, Bolan GA,
Adamsboum C, Jones EE, Phillipa C, Tiendrebeogo H and Yada A.
Age-specific differences in duration of clinical protection after
vaccination with meningococcal polysaccharide A vaccine. Lancet
1985;2:114-117.
- Tauney AD, Galvai PA, de Morais JS, Gotschlich EC and Feldman
RA. Disease prevention by meningococcal serogroup C polysaccharide
vaccine in preschool children. Pediatric Res 1974;8:429.
- Peltola H, Safary A, Kayhty H, Karanko V and Andre FE. Evaluation
of two tetravalent (ACYW135) meningococcal vaccines in infants
and small children- a clinical study comparing immunogenicity
of O-acetyl negative and O-acetyl positive group C polysaccharides.
Paediatrics 1985;76:91-96.
- Lepow ML, Beeler J, Randolph M, Samuelson JS and Hankins WA.
Reactogenicity and immunogenicity of a quadrivalent combined meningococcal
polysaccharide vaccine in children. J Infect Dis1986;154:1033-1036.
- Ceesay SJ, Allen SJ, Menon A, Todd JE, Cham K, Carlone MG,
Turner SH, Gheesling LL, DeWitt W, Plikaytis BD and Greenwood
B. Decline in meningococcal antibody levels in African children
5 years after vaccination and the lack of an effect of booster
immunisation. J lnfect Dis 1993;167:1212-1216.
- Zangwill KM, Stout RW, Carlone GM, Pais L, Harekeh H, Mitchell
S, Wolfe WH, Blackwood V, Plikaytis BD and Wenger JD. Duration
of antibody response after meningococcal polysaccharide vaccination
in US Air Force personnel. J Infect Dis 1994;169:847-852.
- Cruse JM and Lewis RE. Conjugate vaccines. Contrib Microbiol
Immunol 1989;10: 1-196.
- Anderson P, Pichichero M, Edwards K, Porch CR and Insel R.
Priming and induction of Haemophilus influenzae type b capsular
antibodies in early infancy by Dpa20, an oligosaccharide-protein
vaccine. J Pediatr 1987; 11:644-650.
- Constantino P, Viti S, Podda A, Velmonte MA, Nencioni L and
Rappuoli R. Development and phase 1 clinical testing of a conjugate
vaccine against meningococcus A and C. Vaccine 1992;10:691-698.
- Rappuoli R. Conjugate vaccines against meningococcus A, C
and B. In Bacterial meningitis, progress towards elimination.
Winchester, U.K.:Meningitis Trust 1993.
- Broome CV. Group A meningococcus: Epidemiology and development
of a protein polysaccharide conjugate vaccine. In: Neisseria 1990.
Eds. Achtman M, Kohl P, Marchal C, Morelli G, Seiler A and B.
Thiesen. Walter de Gruyter. Berlin 1991;17-24.
- Finne J, Leinonen M and Makela PH. Antigenic similarities
between brain components and bacteria causing meningitis. Lancet
1983;ii:355-257.
- Zollinger WD, Mandrell RE, Griffiss JM, Atieri P and Berman
S. Complex of meningococcal group B polysaccharide and type 2
outer membrane protein immunogenic in man. J Clin Invest 1979;63:836-848.
- Jennings HJ and Lugowski C. Immunochemistry of groups A, B
and C meningococcal polysaccharide-tetanus toxoid conjugates.
J Immunol 1981;127:1011-1018.
- Frasch CE, Peppler MS, Cate TR and Zahradnik JM. Immunogenicity
and clinical evaluation of group B Neisseria meningitidis outer
membrane protein vaccines. In: Seminars in infectious diseases.
IV. Bacterial vaccines 1982);Eds.Robbins JB, Hill JC and Sadoff
JC. Thieme-Stratton. New York:263-267.
- Lifely MR, Roberts SC, Shepherd WM, Esdale J, Wang Z, Cleverly
A, Aulaqi A and Moreno C. Immunogenicity in adult males of a Neisseria
meningitidis group B vaccine composed of polysaccharide complexed
with outer membrane proteins. Vaccine 1991; 9:60-65.
- Jennings HJ, Roy R and Gamian A. Induction of meningococcal
group B polysaccharide-specific IgG antibodies in mice using an
N-propionylated B polysaccharidetetanus toxoid conjugate vaccine.
J Immunol 1986;137:1708-1713.
- Jennings HJ, Gamian A and Ashton FE. N-Propionylated group
B meningococcal polysaccharide mimics an unique epitope on group
B Neisseria meiningitidis. J Exp Med 1987;165:1207-1211.
- Jennings HJ, Gamian A, Michon F and Ashton FE. Unique intermolecular
bactericidal epitope involving the homosialopolysaccharide capsule
on the cell surface of group B Neisseria meningitidis and Escherichia
coli Kl. J Immunol 1989;142:3585-3591.
- Mandrell RE and Zollinger WD. Human immune response to meningococcal
outer membrane protein epitopes after natural infection or vaccination.
Infec Immun 1989;57:1590- 1598.
- Wedege E, Bjune G, Froholm LO, Hoiby EA and E. Rosenqvist.
Immunoblotting studies of vaccinee and patient sera from a Norwegian
serogroup B meningococcal vaccination trial. NIPH Ann 1991;14:183-186.
- Orren A, Warren RE, Potter PC, Jones AM, Lachman PJ and Poolman
JT. Antibodies to meningococcal class 1 outer membrane proteins
in South African Complement-deficient and Complement-sufficient
subjects. Infect Immun 1992;60:4510-4516.
- Klugman KP, Gotschlich E C and Blake MS. Sequence of the structural
gene (rmpM) for the class 4 outer membrane protein of Neisseria
meningitidis, homology of the protein to gonococcal protein III
and Escherichia coli Omp A and construction of meningococcal strains
that lack class 4 protein. Infect Immun 1989;57:2066-2071.
- Munkley A, Tinsley CR, Virji M and Hechels JE. Blocking of
bactericidal killing of Neisseria meningitidis by antibodies directed
against class 4 outer membrane protein. Microb Pathog 1991;11:447-452.
- White DA, Barlow AK, Clarke IN and Heckels JE. Stable expression
of meningococcal class 1 protein in an antigenically reactive
form in outer membranes of Escherichia coli. Mol Microbiol 1990;4:769-776.
- Nurminen M, Butcher S, Idanpaa-Heikkila I, Wahlstrom E, Muttilainen
S, Runeberg-Nyman K, Sarvas M and Makela PH. The class 1 outer
membrane protein of Neisseria meningitidis produced in Bacillus
subtilis can give rise to protective immunity. Mol Microbiol 1992;6:2499-2509.
- Poolman JT, Van der Ley PA, Wiertz EJHJ and HP. Second generation
meningococcal OMP-LPS vaccine. NlPH Ann 1991;14:233-241.
- Van der Ley P and Poolman JT. Construction of a multivalent
meningococcal vaccine strain based on the class 1 outer membrane
protein. Infec Immun 1992; 60:31563 161.
- McCarvil J, McKenna AJ, Grief C, Hoy CS, Sesardic D, Maiden
MCJ and Feavers I M. Expression of meningococcal epitopes in LamB
of Escherichia coli and the stimulation of serosubtype-specific
antibody responses. Mol Microbiol 1993;10: 203-213.
- Bjune G, Hoiby EA, Gronnesby JK, Arnesen O, Fredriksen JH,
Halstensen A, Holten E, Lindbak AK, Nokleby H, Rosenqvist E, Solberg
LK, Closs O, Eng J, Froholm LO, Lystad A, Bakketeig LS and Hareide
B. Effect of outer membrane vesicle vaccine against group B meningococcal
disease in Norway. Lancet 1991;338:1093-1096.
- Fredriksen JH, Griffiths E, Grotterod EM, Hoiby EA, Rosenqvist
E, Stevenson P and Wedege E. Characterization of high molecular
weight components in Men B-vaccine Folkhelsa: an outer membrane
vesicle vaccine against group B meningococcal disease. In: Pathobiology
and immunobiology of Neisseriaceae. Eds. Conde GCJ, Morse S, Rice
P, Sparling F and Caldern E. Publications office of the Instituto
Nacional de Salud Pblica. Cuernavaca, Mexico 1994; 818-824.
- Griffiths E, Sierra G and Holst J. Quality control of the
Cuban and Norwegian serogoup B vaccines used in the Iceland study.
In: Neisseria 1994. Eds. Evans JS, Susan EY Martin CJM and Feavers
IM. NIBSC. Potters Bar UK.1994;437.
- Perkins BA, Jonsdottir K, Briem H, Plikaytis BD, Griffiths
E, Hoiby EA, Rosenqvist E, Holst J, Nokleby H, Sotolongo F, Sierra
G, Huergo C, Carlone G, Williams D, DykesJ, Weneger JD and Broome
CV. Comparison of two outer membrane protein-based serogroup B
meningococcal vaccines and effect of a booster dose among young
adults in Reykjavik, Iceland. In: Neisseria 94. Eds. Evans JS,
Susan EY, Martin CJM and Feavers IM. NIBSC. Potters Bar, UK.1994;438-439.
- Ala'Aldeen DAA and Griffiths E. Vaccine development against
meningococcal disease. In: Molecular and clinical aspects of bacterial
vaccine development. Eds. Ala'Aldeen DAA and Hormaeche CE. John
Wiley and Sons Ltd. Chichester 1995;1-39.
- Schryvers AB and Morris LJ. Identification and characterization
of the transferrin receptor from Neisseria meningitidis. Mol Microbiol
1988; 2:281-288.
- Ala'Aldeen DAA, Stevenson P, Griffiths E, Gorringe AR, Irons
LI, Robinson A, Hyde S and Borriello SP. Immune responses in man
and animals to meningococcal transferrin-binding proteins: implications
for vaccine design Infect Immun 1994;62: 2894-2900.
- Schryvers AB and Morris LJ. Identification and characterization
of the human lactoferrin-binding protein from Neisseria meningitidis.
Infect Immun 1988a;56:11441 149.
- Chen CY, Berish SA, Morse SA and Mietzner TA. The ferric iron-binding
protein of pathogenic Neisseria spp. functions as a periplasmic
transport protein in iron acquisition from human transferrin.
Mol Microbiol 1993; 10:311-318.
- Lewis LA and Dyer DW. Identification of an iron-regulated
outer membrane protein of Neisseria meningitidis involved in the
utilization of hemoglobin complexed to haptoglobin. J Bacteriol
1995;177:1299-1306.
- Thomson SA and Sparling PF. Cytotoxin-related FrpA protein
of Neisseria meningitidis is excreted extracellularly by meningococci
and by Hly BD+ Escherichia coli. Infect Immun 1993;61:2908-2911.
- Thomson SA, Wang LI, West A and Sparling PF. Neisseria meningitidis
produces iron-regulated proteins related to the RTX family of
exoproteins. J Bacteriol 1993;175:811-818.
- Ala'Aldeen DAA, Wall RA and Borriello SP. Immunogenicity and
cross-reactivity of the 70-KDa iron-regulated protein of Neisseria
meningitidis in man and animals. J Med Microbiol 1990;32:275-281.
- Ala'Aldeen, DAA, Davies HA and Borriello SP. Vaccine potential
of meningococcal FrpB: studies on surface exposure and functional
attributes of common epitopes. Vaccine 1994;12:535-541.
- Ala'Aldeen DAA, Powell NBL, Wall RA and S. P. Borriello. Localization
of the meningococcal receptors for human transferrin. Infect Immun
1993;61:751-759.
- Lissolo LC, Dumas Maitre-Wilmotte P, Mignon M, Danve B and
Quentin-Millet M-J. Evaluation of transferrin-binding protein
2 within the trasferrin-binding complex as a potential antigen
for future meningococcal vaccines. Infect Immun 1995;63:884-89
- Rokbi B, Mazarin V, Maitre-Wilmotte G and Quentin-Millet MJ.
Identification of two major families of transferrin receptors
among Neisseria meningitidis strains based on antigenic and genomic
features. FEMS Microbiol Lett. 1993;110:51-58.
- Ala'Aldeen DA, Davies HA, Wall RA and Borriello SP. The 70
kilodalton iron regulated protein of Neisseria meningitidis is
not the human transferrin receptor. FEMS Microbiol Lett 1990;69:37-42.
- Griffiths E, Stevenson P and Ray A. Antigenic and molecular
heterogeneity of the trasferrin-binding protein of Neisseria meningitidis.
FEMS Microbiol Lett 1990;69:31-36.
- Legrain M, Mazarin V, Irwin SW, Bouchon B, Quentin-Millet
MJ, Jacobs E and Schryvers AB. Cloning and characterization of
Neisseria meningitidis genes encoding the transferrin-binding
proteins Tbp 1 and Tbp 2. Gene 1993;130:73-80.
- Stevenson P, Williams P and Griffiths E. Common antigenic
domains in transferrin-binding protein 2 of Neisseria meningitidis,
Neisseria gonorrhoeae, and Haemophilus influenzae type b. Infect
Immun 1992;60:2391-2396.
- Ferreirs CM, Ferrn L and Criado MT. In vivo human immune response
to transferrin-binding protein 2 and other iron-regulated proteins
of Neisseria meningitidis. FEMS Immunol Med Microbiol 1994;8:63-68.
- Danve B, Lissolo L, Mignon M, Dumas P, Colombani S, Schryvers
AB and Quentin-Millet M. Transferrin-binding proteins isolated
from Neisseria meningitidis elicit protective and bactericidal
antibodies in laboratory animals. Vaccine. 1993;11:1214-1220.
- Ala'Aldeen DAA and Borriello SP. The meningococcal transferrin-binding
proteins 1 and 2 are both surface exposed and generate bactericidal
antibodies capable of killing homologous and heterologous strains.
Vaccine, In press.
- Bishop K, Ala'Aldeen DAA, Powell NBL, Gorringe AR and Borriello
SP. Detection of linear and conformational epitopes on the TBP1
component of the meningococcal transferrin receptors, using monoclonal
antibodies. J. Med. Microbiol 1995;42:148.
Copyright 1996 Elfos Scientiae
|